When the pioneering science fiction writer Jules Verne wrote Journey To The Centre Of The Earth in 1864, he probably knew that his plot was pure fantasy. Verne’s characters only made it a few miles down but the idea that anyone could even contemplate travelling to the Earth’s core had been dismissed before Victorian times.
In fact even today, the furthest we’ve drilled into the Earth is around 12km, while the distance to the centre is over 500 times further, at 6,370km.
So how do we know what lies beneath? Figuring out what’s at the heart of our planet has been a magnificent scientific puzzle.
How do we know the Earth is round?
The idea of the Earth having a meaningful centre goes hand-in-hand with the planet being shaped like a ball, and we’ve known that we don’t live on a disc for a long time.
It’s a myth that medieval folk thought the Earth was flat – this actually came from a mix of Victorian anti-religious propaganda, and a misinterpretation of the stylised maps of the period.
It was over 2,200 years ago that the Greek polymath Eratosthenes made the first measurement of the distance around the Earth’s sphere, and it’s been clear ever since that it must have a centre.
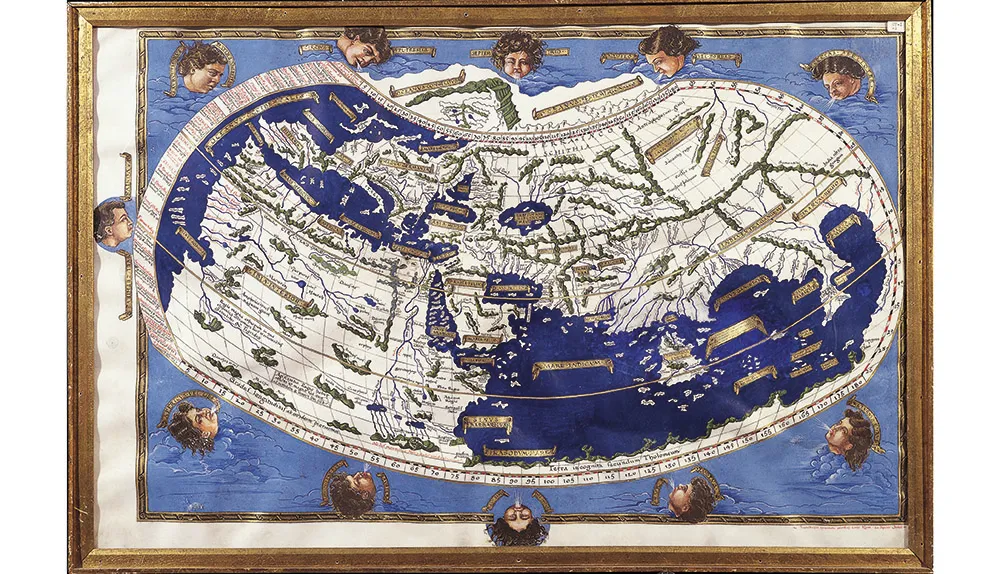
This doesn’t mean, though, that early philosophers thought of Earth as we do today.
Ancient Greek physics said that the world consisted of a series of concentric spheres of four fundamental elements: earth, water, air and, finally, fire.
In this oldest scientific picture, the centre of the planet had to be solid, as air couldn’t be inside the sphere of earth.
Clearly, the sphere of earth wasn’t completely surrounded by water or there’d be no dry land, so there was thought to be a bit of the earth sticking out – meaning there could be only one continent.
As a result, the discovery of the Americas was, in fact, one of the first experimental scientific results, disproving the idea of a single continent, and marking a significant step on the way to moving beyond Ancient Greek scientific thinking.
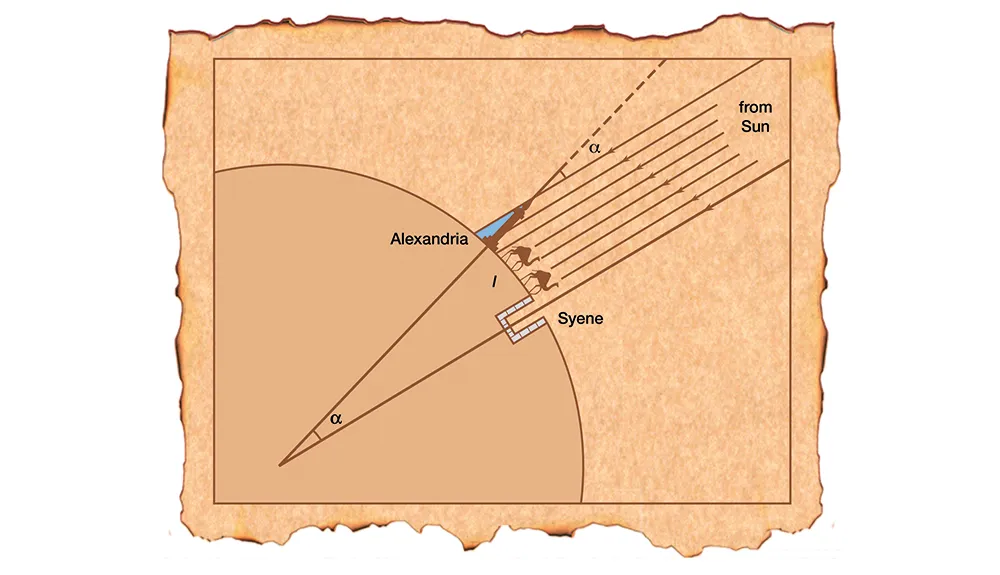
The idea of the Earth being entirely hollow, or with vast caverns reaching to the centre as in Verne’s book, has been popular in fiction and mythology since ancient times, also featuring in pseudoscience and conspiracy theories.
However, it’s not clear that any scientist apart from the astronomer Edmond Halley, who proposed a hollow Earth to explain some unusual compass readings in 1692, has ever taken this idea seriously.
And in 1798, an English scientist and eccentric put the final nail in the coffin of the ‘hollow Earth’ hypothesis. Step forward Henry Cavendish, with an experiment to accurately weigh the planet.
How much does Earth weigh?
Cavendish was an odd man, who only communicated with his servants via notes to avoid meeting them face-to-face.
Despite his aristocratic background, Cavendish dedicated his life to science, working in both chemistry and physics, and most famously devised an experiment to calculate the density of the Earth.
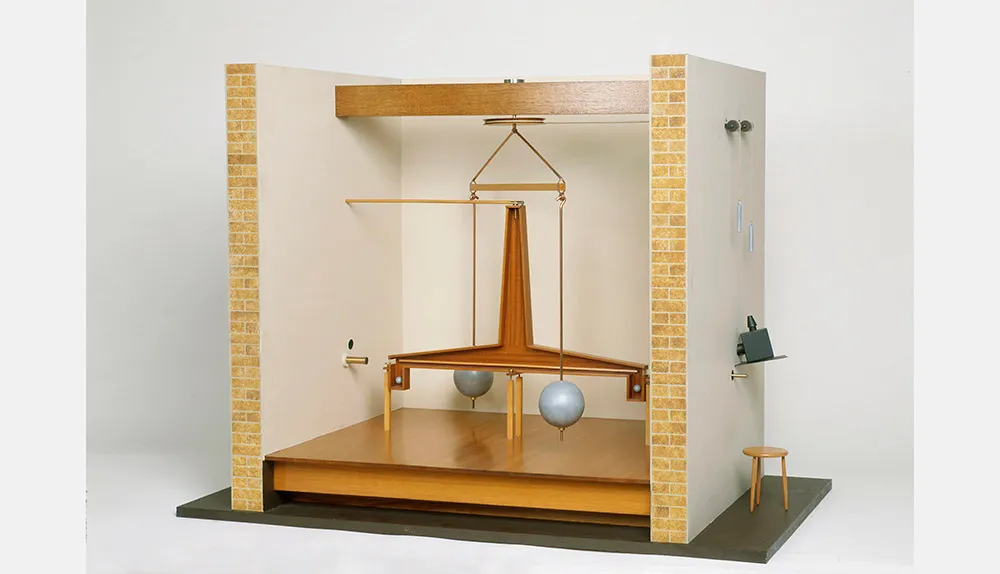
Using a simple torsion balance, which measured the amount of twisting force caused by the gravitational pull of two large balls on a smaller pair, Cavendish was able to calculate the faint gravitational attraction between the two pairs of balls.
By comparing this with the Earth’s own gravitational pull, he could work out the planet’s density (and, as the Earth’s size was already known, its mass, too).
But the density figure showed that our planet must be mostly solid, unless there were extremely dense unknown materials somewhere in the depths.
How do we know what's at the Earth's core?
Today, we split the innards of the Earth into three segments: the crust, which is the outer layer, between 5km and 75km thick, the mantle, extending to a depth of around 2,900km, with the thickness of the core – the bit we’re interested in here – extending around 3,500km out from the Earth’s centre, with two distinct segments.
At the core’s heart is an extremely hot but still solid nickel-iron sphere with a radius of around 1,200km. At approximately 5,400°C, this inner core is similar in temperature to the surface of the Sun. The remainder is the Earth's liquid outer core, made of mostly nickel-iron, with similar temperatures, getting hotter towards the centre.
But how can we possibly know such detail about a location that is so inaccessible?
Given the near-impossibility of ever getting even within a thousand kilometres of the core, all our knowledge is indirect and depends on seismology – the science of earthquakes.
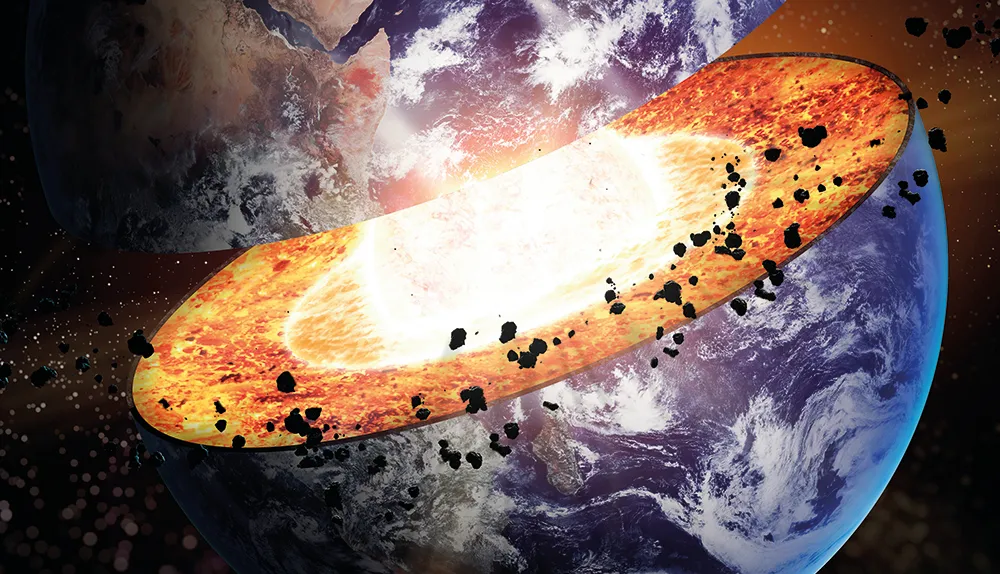
After a quake, seismic waves travel through the Earth, changing their form and direction depending on the materials they pass through. Geophysicists have used this information to deduce what lies at the Earth’s core.
Their seismometers, devices to measure such waves, are the equivalent of telescopes for exploring the Earth’s interior.
Read more about the Earth's core:
- What will happen if the Earth's core cooled down?
- The Earth's magnetic field reverses more often – now we know why
- Could we send a robotic probe to Earth’s core?
- Does the Moon have a molten core?
By the early 20th Century, the increasing temperatures as we dug deeper into the Earth, combined with seismologists’ analysis of earthly waves, suggested that the inner parts of our planet were at least partly molten – hot enough to turn rock and metal into liquid.
And the key discoveries were made by two scientists who, shamefully, were never even nominated for a Nobel Prize: British geologist Richard Oldham and Danish seismologist Inge Lehmann.
What can waves tell us about Earth's structure?
Think of a wave, and you’ll probably think of a surface wave, like one you’d see on the sea. But many waves – sound, for example – travel through the body of a material.
Though the seismic waves that cause damage in an earthquake are those that travel on the surface, there are also two types of ‘body wave’ that move through the Earth. P-waves (‘P’ stands for ‘primary’) are longitudinal waves, just like sound.
They vibrate in the direction of movement, causing the Earth to squash up and expand as they pass through.
P-waves travel rapidly – around 5km per second in a rock like granite, and up to 14km per second in the densest parts of the mantle.
The second type of body wave, S-waves (‘S’ stands for ‘secondary’), are slower, transverse waves, moving from side-to-side. Unlike P-waves, they can’t travel through a liquid, which is why these two types of wave proved essential in helping us understand the Earth’s core.
Imagine there’s a huge earthquake. Waves begin to move through the Earth.
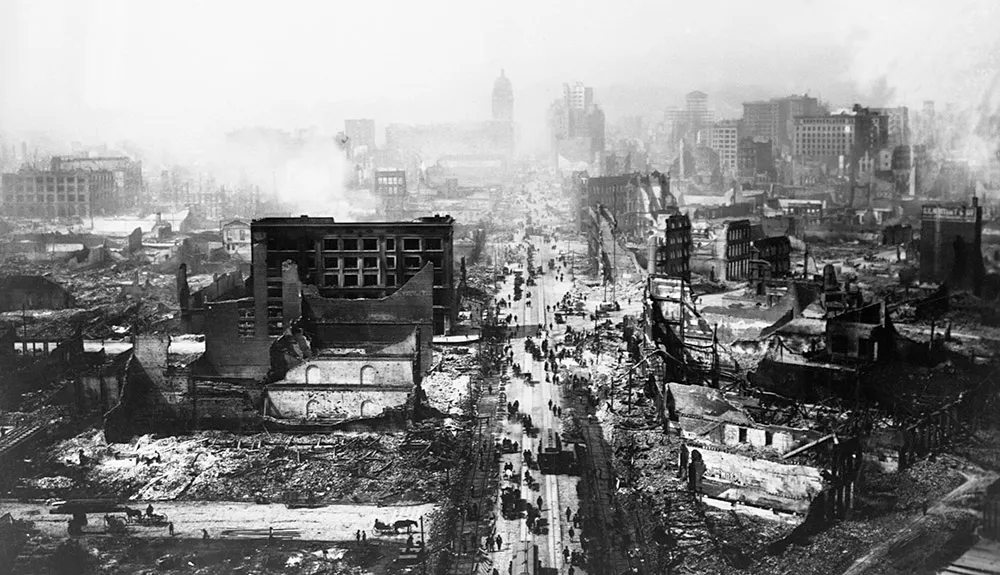
The P-waves shoot ahead, while the S-waves follow behind at around half the speed. Both types of wave will be detected by seismometers, which are used to measure vibrations in the ground, all over the Earth.
But where the waves pass through the core to reach a distant measuring station, there is a so-called shadow zone. Travel about 104° around the Earth’s perimeter from the quake’s epicentre and the waves disappear. But from 140° onwards, the P-waves reappear, with no accompanying S-waves.
As early as 1906, Richard Oldham realised the implications of this odd shadow. Oldham spent most of his career with the Geological Survey of India, often working in the Himalayas.
Read more about earthquakes:
- Can animals help us predict earthquakes?
- How to stop an earthquake
- What is a magnitude 10 earthquake like?
- Flat plates linked to mega earthquakes
When he retired to the UK in 1903, he made use of the data accumulated over the previous few years to probe the interior of the Earth. He realised that the observed P-wave and S-wave behaviour could be explained if the centre of the Earth was liquid.
In such a case, P-waves would be refracted by the liquid, bending as light does when it moves from water to air, leaving a distinctive shadow. S-waves, by contrast, would be stopped entirely by a liquid core.
Oldham’s breakthrough led to a widely accepted picture of a molten core, but 30 years later, Inge Lehmann realised that Oldham’s idea was too simple.
The refraction of the P-waves by the dense liquid in the centre of the Earth should have produced a total shadow.
In fact, measurements made with the more sensitive seismometers available by Lehmann’s time showed that faint P-waves were still arriving in the shadow zone.
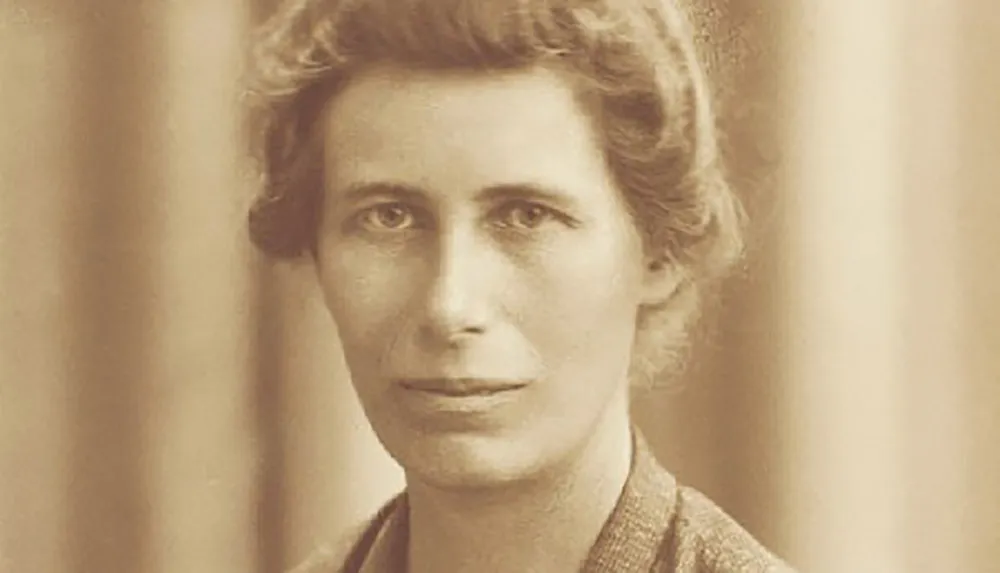
By studying data passing through the planet from a 1929 New Zealand earthquake Lehmann proposed that these waves were being reflected off the boundary between an inner solid core and the outer liquid.
Her results, published in 1936, were confirmed two years later by Beno Gutenberg and Charles Richter, who accurately modelled the effects of a solid core.
Direct measurements of these reflected seismic waves finally came in 1970.
What is the core of the Earth made of?
Further studies picked up even more subtle waves which, from their delayed arrival, had to have crossed the liquid outer core as P-waves, before being converted to transverse S-waves in the inner core, and then back to P-waves on the way out.
This discovery, only confirmed in 2005, was further proof of the solid core.
Even so, the exact nature of the inner core is subject to significant debate. Temperatures, for instance, can only be worked out from experimental studies of how materials melt and solidify under pressure.
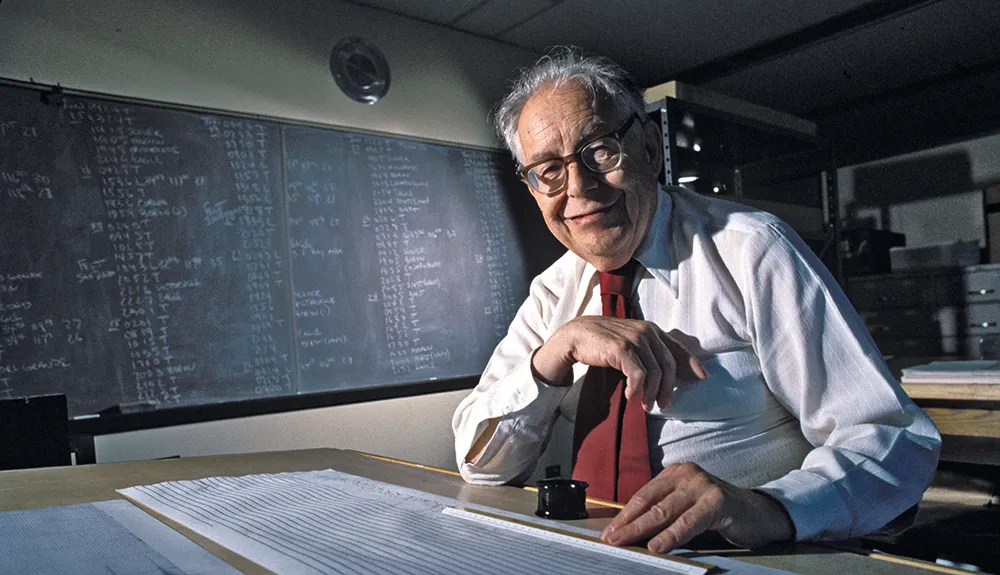
In fact the assumption that the core consists primarily of iron and nickel comes from a combination of the frequency with which different elements occur in our local region of the Milky Way, and our understanding of how our planet formed.
Under the immense pressure at the centre of the Earth – over three million times atmospheric pressure – materials can act very differently from normal conditions.
While the most obvious contender for the inner core is a solid nickel-iron alloy, it is possible for an extremely dense plasma – the state of matter found in a star – to have similar properties. One of the difficulties here is knowing how materials behave in such extreme environments.
Enter the diamond anvil cell.
In this remarkable device, the points of two diamonds, just a fraction of a millimetre across, are squeezed together.
Applying a force to a small area produces more pressure than applying it to a wide one – that’s why being trodden on by a stiletto heel is much more painful than a flat sole.
The diamond anvil creates pressures up to twice that of the Earth’s core, and heating is applied using lasers.
When metallic samples are crushed and heated to core-like conditions, the results suggest a crystalline solid in the centre of the Earth.
Realistically, we will never get anywhere near the Earth’s core.
The levels of heat, pressure and radioactivity (one of the main sources of internal heating) are so high that even if we could bore through over 6,000km of rock and metal, a probe would be unable to survive.
Compared with reaching the core, travelling to the outer reaches of the Solar System is trivial.
But our planet’s own vibrations, produced by earthquakes and interpreted by scientists as ingenious as Inge Lehmann, give us the means to explore with our minds where we will never visit in person.
- This article first appeared in issue 304 of BBC Focus
Key terms
Longitudinal waves - These waves consist of a series of compressions and relaxations in the direction of travel, like a slinky spring given a push along its length. Examples include sound and P-waves.
Refraction - When a wave hits the boundary between two materials, travelling at an angle, it changes direction. Light waves, for instance, are refracted when passing between water and air, making a straight object appear bent.
Seismology - The study of earthquakes. Analysis of how different types of seismic wave travel through the Earth has allowed us to piece together our planet’s inner structure.
Torsion balance - This apparatus consists of a bar, suspended from a frame by a twisty fibre. As the bar moves sideways, it applies a force to the fibre – the further it twists, the greater the force.
Transverse waves - These waves consist of a series of side-to-side vibrations, like the waves sent through a rope by moving one end up and down. Examples include light and S-waves.